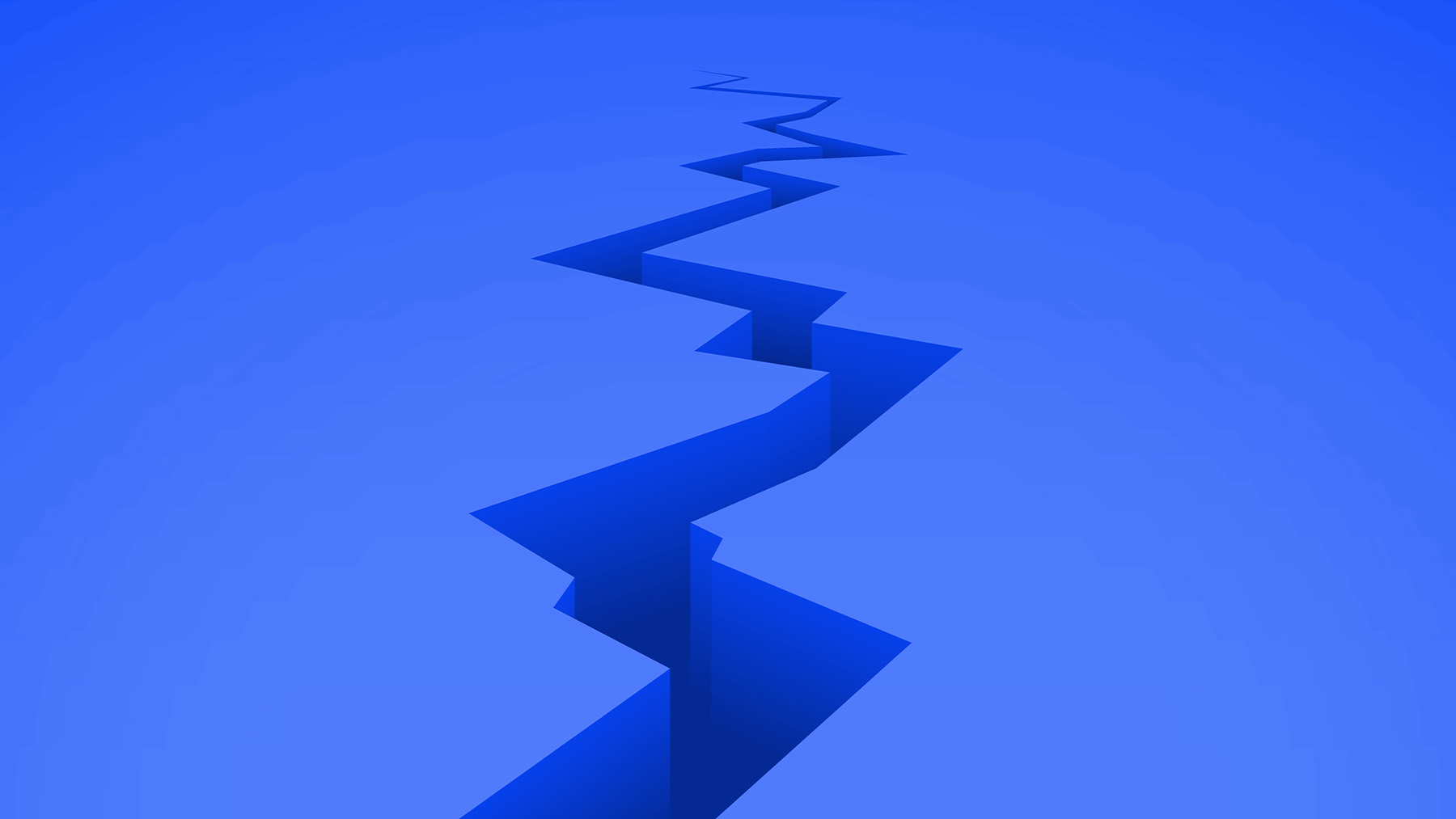
By Justin D. Marshall, Ph.D., P.E., M.ASCE
Recently I listened to an episode of the podcast Cautionary Tales during which host Tim Harford discussed the 1981 Hyatt Regency Hotel walkway collapse in Kansas City, Missouri — a disaster I’d first heard about as an undergraduate. A surprising aspect Harford related was the immediate post-collapse search and rescue effort highlighting the human cost. It struck me deeply that while I had thoroughly contemplated the engineering impacts, the effects on the people had escaped me.
What’s more, being part of post-disaster engineering reconnaissance teams for the 2010 Haiti earthquake and Hurricane Dorian in the Bahamas in 2019 showed me that the “human” lessons we learn from significant natural hazards cannot be gleaned through laboratory testing or detailed computer analyses.
The foundation of our society is intertwined with the built environment. As structural engineers, we design infrastructure that enables the economic and social lifestyles of our communities. People take for granted that engineers do their jobs correctly. However, this societal view of structural engineering is maintained only until it is not.
Design and construction standards ensure the built environment has a minimum level of safety in the face of normal or extreme events that a community may experience. Those standards designate a maximum probability of failure, given a design-level event. But the real impact of these events on a community is measured in terms of loss of life, livelihood, and property.
The code-required minimum design for earthquake resistance is based on balancing economy and life safety. Structural engineers design lateral systems with certain components intended to absorb earthquake energy through inelastic response (damage) to prevent collapse.
As evidenced by comparing the number of fatalities between the 2010, 7.0 magnitude Haiti earthquake (more than 200,000) and the 2011, 6.2 magnitude Christchurch, New Zealand, earthquake sequence (185), a ductility-based seismic design process that is enforced by codes, standards, and inspections is successful at providing life safety, and with a few notable exceptions, the life-safety performance objective was fulfilled in Christchurch.
From my perspective, the movement toward improving infrastructure resilience to seismic events began after the Christchurch earthquake. The local citizens were surprised to learn that the damage done to their engineered buildings had been expected. While many of the damaged buildings were able to be repaired, many were not.
In Christchurch’s central business district alone more than 1,400 buildings were demolished. At the time of the earthquake, the population of Christchurch was approximately 350,000. For larger cities like San Francisco, Los Angeles, or Seattle, the number of buildings that would be damaged and then require either very long and costly repair or demolition would be much greater.
The challenge to structural engineers moving forward is designing structures that meet the life-safety requirement and that are quickly returned to partial or full functionality. As with any challenge, a key first step is defining the problem.
Shifting design strategy
Earthquake damage is classified as either structural or nonstructural. Structural damage includes damage to the beams, columns, shear walls, and foundations. Nonstructural damage includes the cladding and interior finishes as well as the mechanical, electrical, and plumbing services that maintain operations. For manufacturing plants, laboratories, or hospitals, the equipment needed for operation also falls into this category.
Damage to either the structural or nonstructural components can lead to significant repair costs and downtime after a major event. This is where resilience comes into play. The technology and knowledge are available to design structures for life-safety and functional recovery.
How can we integrate this resilience into our design decisions while meeting our clients’ need for economy?
Step 1 — Functional recovery objectives
The first aspect of resilient design is to establish functional recovery objectives. These objectives are particular to the occupants’ and owner’s requirements. Building codes and standards (e.g., the International Building Code and ASCE 7) affect these objectives through mandated minimum life-safety requirements.
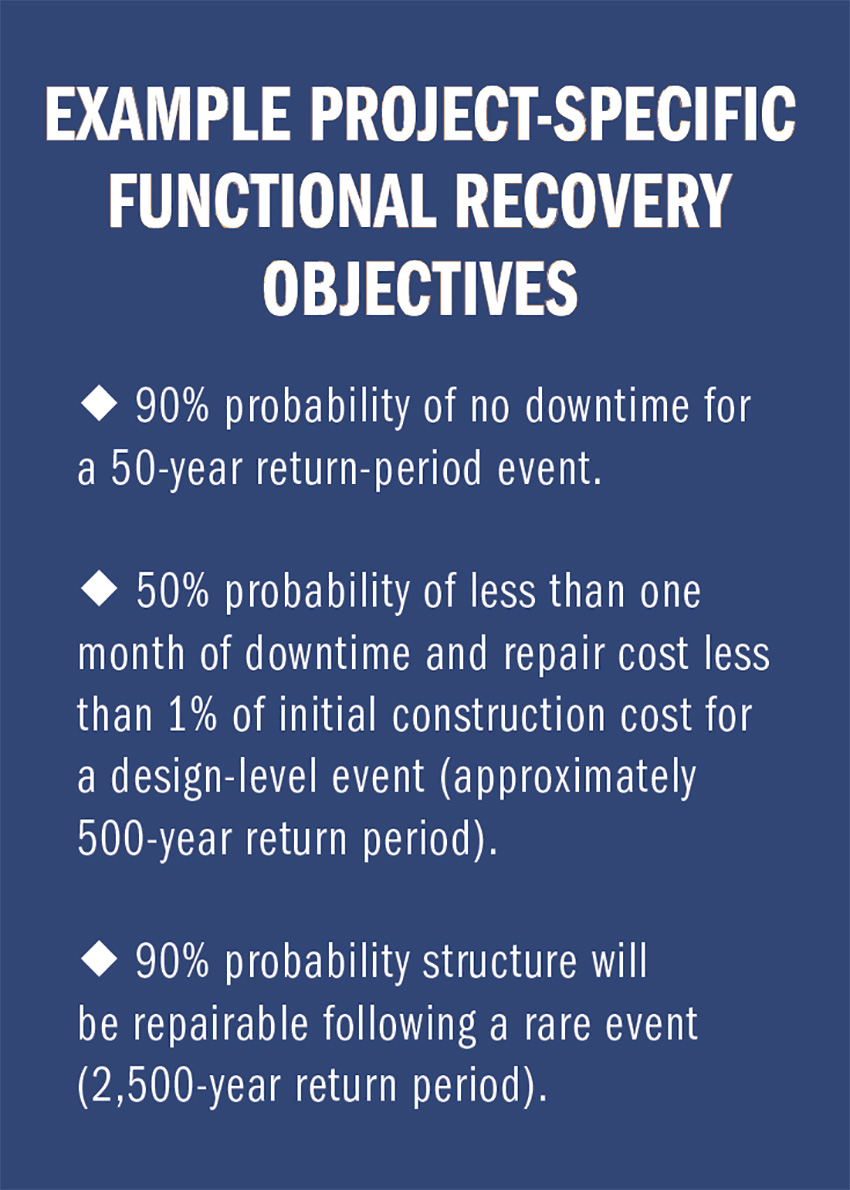
For more important structures (hospitals, emergency operation centers, etc.), increased design requirements improve performance and post-event recovery. The building code requires minimum standards but does not prevent designing to a higher standard.
Structural engineers should initiate discussions related to the functional recovery objectives early in the design process to ensure that the owner and the design team are aware of the expected damage from a significant seismic event. During these discussions it is vital to define resilience objectives that are consistent with the project budget.
Engineers should clearly communicate that meeting a code minimum will result in damage that could require repairs that render the building partially or completely unoccupiable for months to years following an event.
Repair costs and downtime are consequences to be considered in functional recovery objectives. A simple example of a functional recovery objective would be a 50% probability of downtime of less than one month following a design-level earthquake. There could also be conditions on the repair cost. A more detailed example of functional recovery objectives can be seen above. Note that the objectives are based around a given event and a probability expectation.
Step 2 — Lateral system selection
The functional recovery objectives are critical in selecting the lateral and gravity systems. Different lateral systems (shear walls, braced frames, moment frames) have different functional recovery characteristics. Very stringent functional recovery objectives may require the use of seismic isolation or supplemental viscous dampers.
Shear walls and braced frames tend to have lower relative story drifts, but they also have higher floor accelerations. Moment frames tend to have higher relative story drifts but lower floor accelerations. Both structural and nonstructural damage are affected by the dynamic characteristics of the lateral system.
High-ductility systems will incur significant damage in a design-level or greater earthquake. The building code (IBC and ASCE 7) provisions that improve functional recovery require designing for higher forces and decreasing allowable drift limits. These requirements improve functional recovery, but they have cost implications. Strength and stiffness are two controls that structural engineers have that could affect functional recovery, but they are not the only controls available.
One way to enhance functional recovery at little or no extra cost is to use a lateral system with a replaceable fuse. Replaceable fuses are designed to absorb earthquake energy in a component that is easily repaired or replaced. Figure 1 shows the difference between a reinforced-concrete shear wall and a coupled reinforced-concrete shear wall.
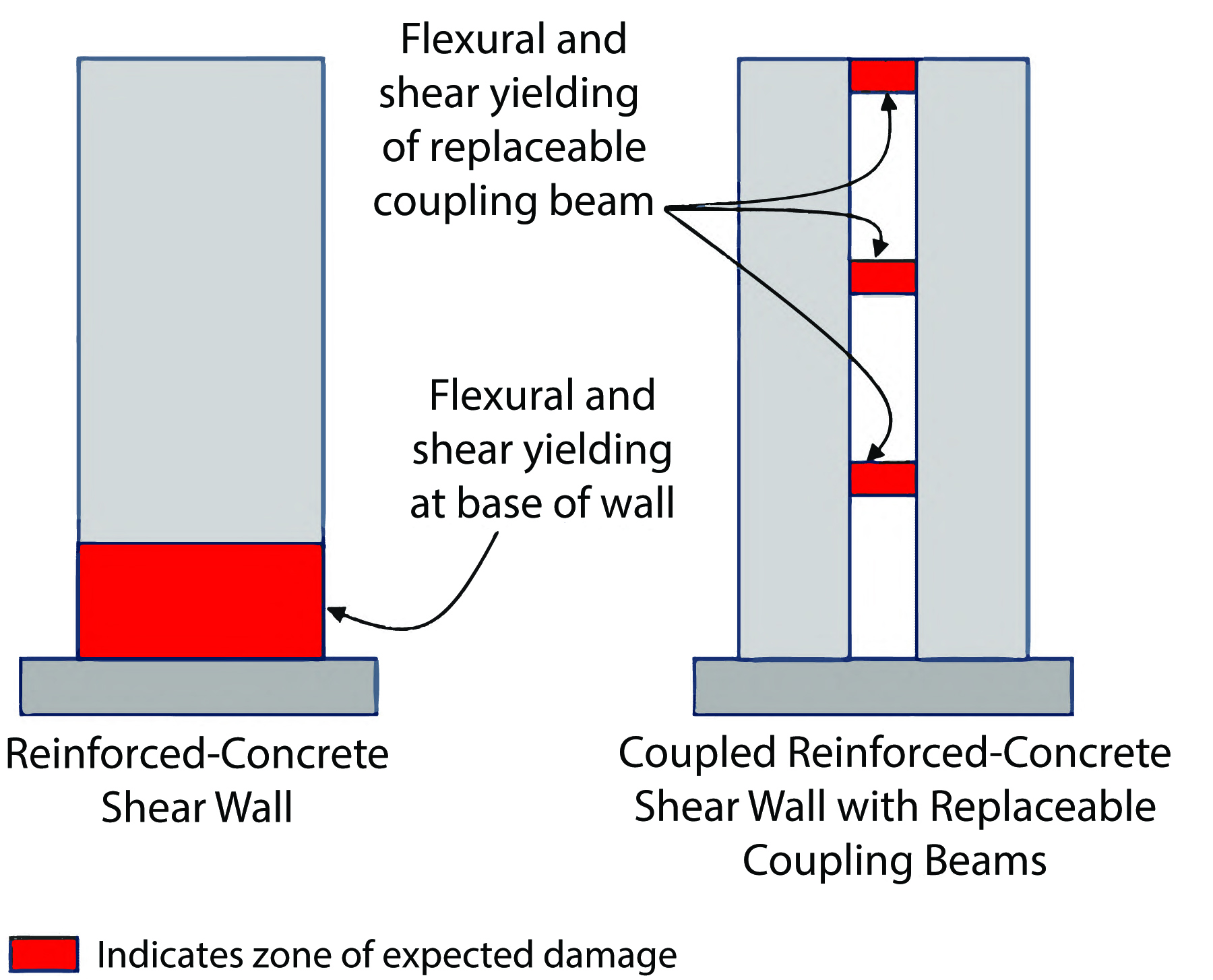
For the coupled wall system, the damage is focused in the coupling beams, which can be repaired or replaced following a significant seismic event. Typical reinforced-concrete walls are designed to yield at the base of the wall, which can be very difficult to repair.
Figure 2 shows a comparison between different steel moment frame connections. Typical design practice is to ensure the beam yields in flexure near the beam-column connection. Following a damaging earthquake, the beam would have to be repaired or replaced, which would be very costly and could require demolition. The beam-column connection can be designed to be easily repairable by providing a yielding fuse plate at the beam bottom flange. The fuse is designed to be easily replaced when damaged in an earthquake.
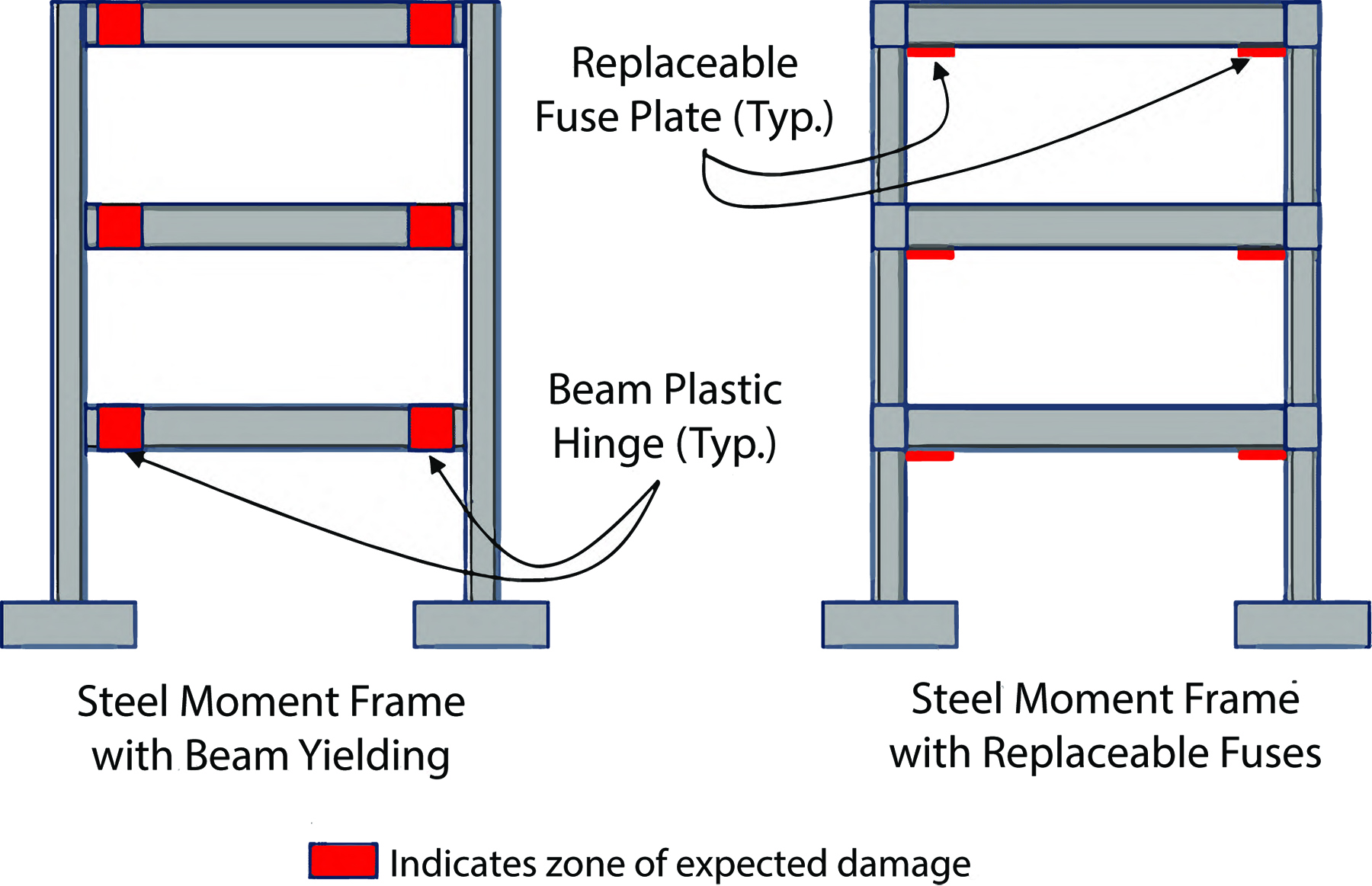
Figure 3 compares three configurations. A conventional concentrically braced frame is designed so that the steel diagonal braces yield in tension and buckle in compression, causing damage to several components of the frame (e.g., braces and gusset plates). A more resilient option is a buckling-restrained braced frame. A buckling-restrained brace is specially designed to yield in tension and compression. The damage is confined to the BRB, which can be replaced following a significant seismic event. BRBs are an economical lateral system that have many performance benefits over a CBF system.
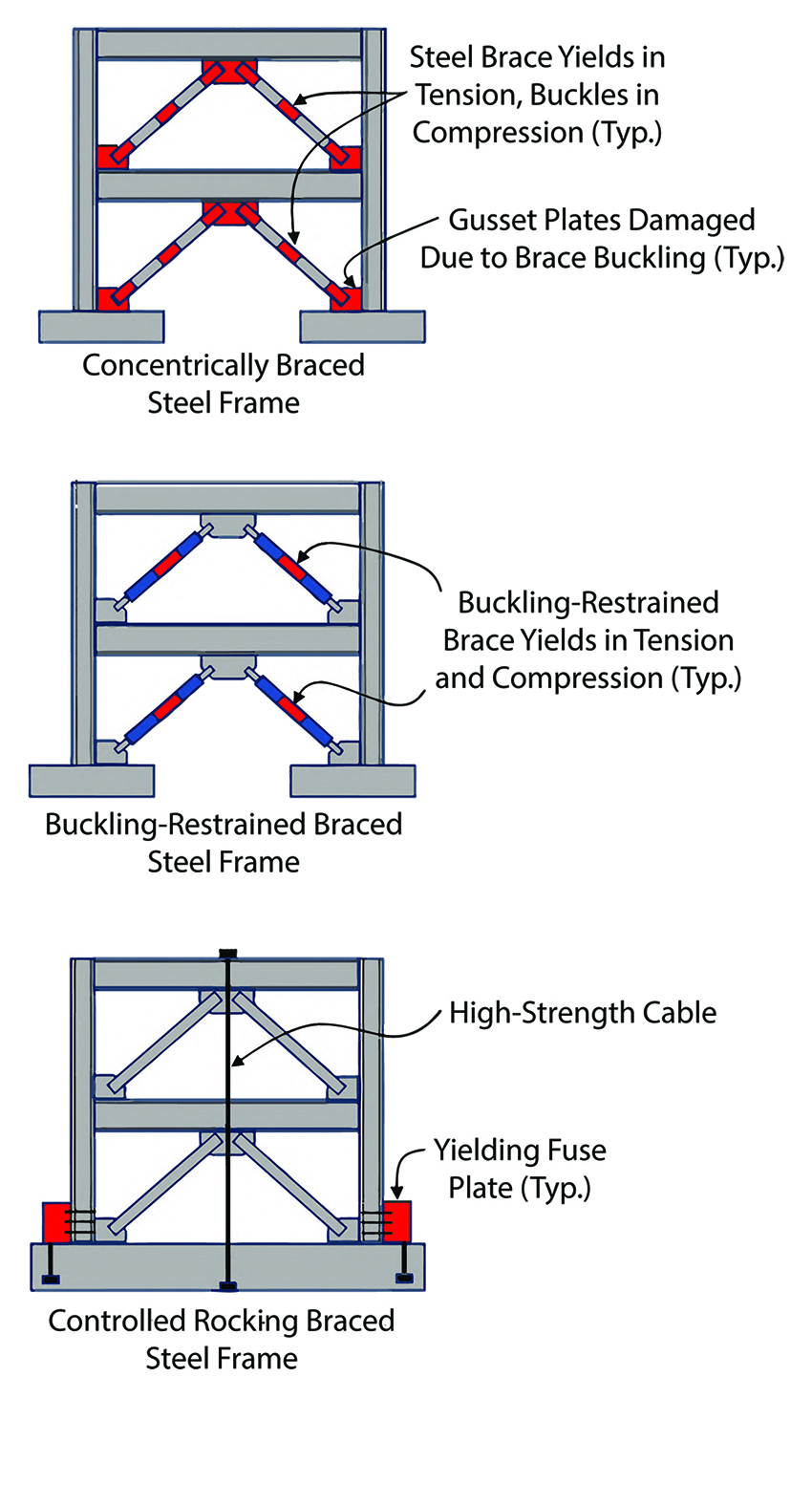
A rocking braced frame is a unique lateral system in which the braced frame is allowed to rock at the foundation level. The example shown in Figure 3 also has the advantage of being able to self-center. The frame recenters itself using a tensioned, high-strength steel cable. The damage is absorbed in the yielding fuses on either side of the frame, which are designed to be easily replaceable. An RBF is likely to increase the initial frame cost, but the benefits to functional recovery after an earthquake would more than offset the upfront costs.
Thinking differently about selecting the lateral system is a critical part of integrating resilience into structural design. Minimizing repair cost and downtime will always improve functional recovery. The probability that the structure will have unrepairable damage and/or require a complete demolition will also decrease significantly.
Structural engineers should move away from thinking of buildings as disposable and instead consider damage mitigation and repairability in our decisions.
Step 3 — Nonstructural components and systems design
Structural engineers should also consider nonstructural systems as a critical aspect of design strategies because these systems have a significant impact on post-event recovery. As mentioned previously, critical nonstructural components can include cladding, interior finishes, MEP services, and the equipment needed for the building to function. Other nonstructural components include signage, stairs, elevators, and furnishings.
In many scenarios, nonstructural components can control the functional recovery design. When looking at the overall cost of a building, the structural system ranges from 8% to 20% of the total building cost, meaning that the nonstructural systems are at least 80% of the budget. Some nonstructural components are considered drift-sensitive, which means that reducing the expected lateral drift during an earthquake will reduce damage and improve functional recovery. This requires designing for greater stiffness.
Other nonstructural components are acceleration-sensitive and respond to an increase in frame stiffness with higher floor accelerations. In this scenario, increasing the stiffness of the structure, which helps with drift-sensitive equipment, would result in more damage to acceleration-sensitive equipment. Finding the right balance is the key to improving functional recovery. Making design decisions about the lateral system (e.g., system type, stiffness, and strength) and detailing for nonstructural components and systems is critical to improving functional recovery.
Assessing functional recovery in the design process
Design is an iterative process and design for functional recovery is no different. Assessing functional recovery throughout the process is critical to integrating resilience into structural design. The Federal Emergency Management Agency’s FEMA P-58 (Development of Next Generation Performance-Based Seismic Design Procedures for New and Existing Buildings) is a tool that can be used for this process.
The FEMA P-58 methodology was initially developed for performance-based seismic design, but it has become a commonly used functional recovery assessment tool. Many design firms are using this method on their projects, and FEMA has funded work to further improve the process. The methodology can be used directly through an executable program called the Performance Assessment Calculation Tool or by using commercially developed programs such as SP3 (by Haselton Baker Risk Group), which provide a user interface that is simpler to apply.
The program output identifies the expected functional recovery timeline and cost with associated probabilities and breaks down which components control the design. These outputs can be used to identify which changes to the structural system and nonstructural design provisions will enable meeting the functional recovery objectives. This iterative process requires communication among the entire design team to ensure objectives and project needs are balanced.
Integrating resilience to make a difference
The construction industry relies heavily on experience and past successes. The challenge going forward is to educate ourselves and our clients on the new tools being developed and implementing them. Functional recovery can be greatly improved by better understanding the controlling mechanisms and finding the available solutions to improve performance.
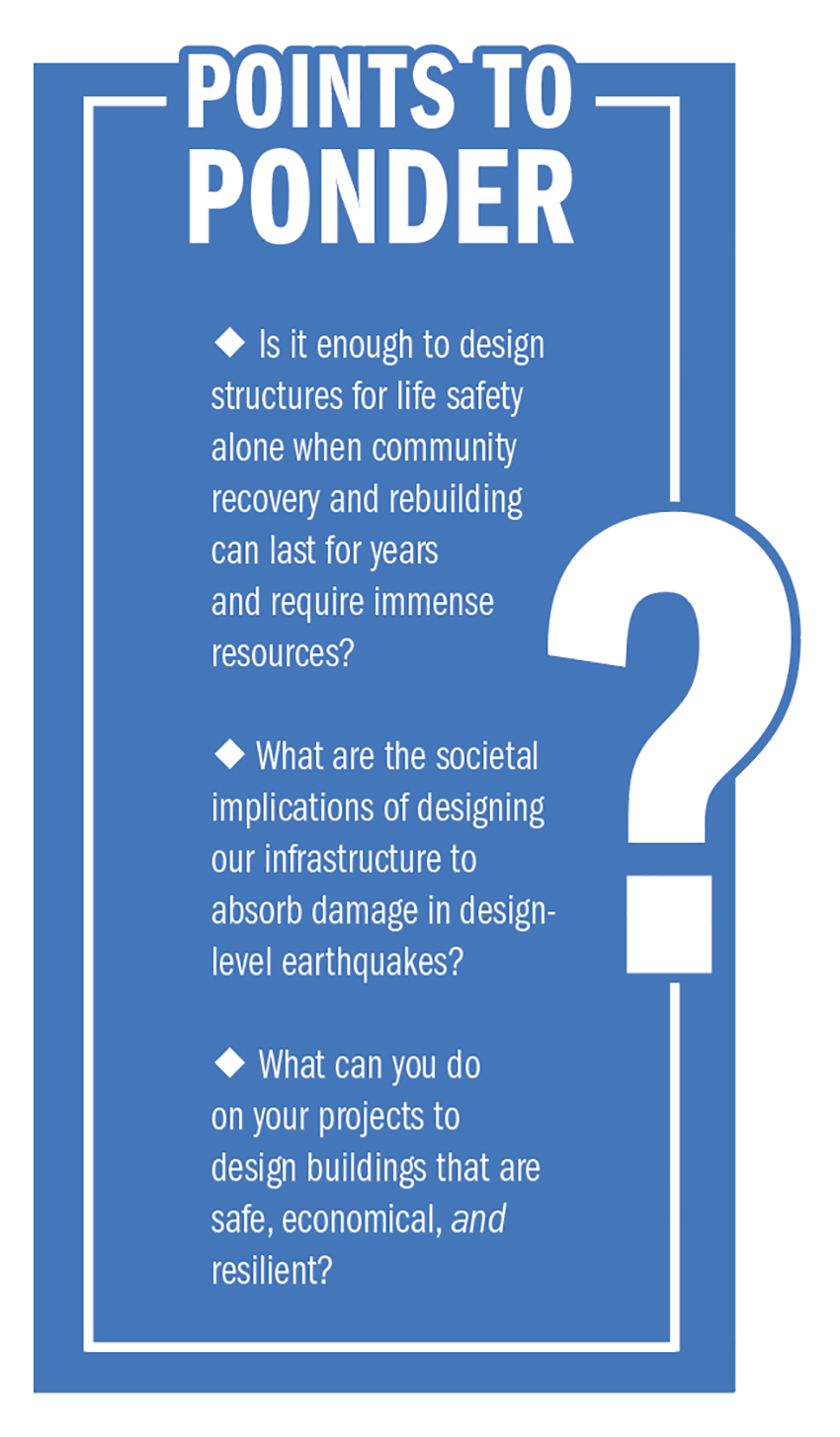
The resilient design process must be integrated within our overall design process. This is accomplished by first communicating with the design team to establish project-specific functional recovery objectives. Second, it involves selecting a structural system that meets these objectives for structural and nonstructural systems. Third, resilient design requires determining the nonstructural system design strategies that will ensure compliance with functional recovery objectives. Lastly, it requires assessing building performance throughout design using the FEMA P-58 methodology.
Our profession is critical to the infrastructure our society requires. Meeting life-safety demands and ensuring our buildings are not disposable in earthquakes requires consideration of functional recovery. To do this we must think differently about design. Change is not easy, but we can be the ones to drive it.
References
ASCE. (2022). Minimum Design Loads and Associated Criteria for Buildings and Other Structures, ASCE/SEI 7-22. ASCE, Reston, Virginia.
Federal Emergency Management Agency. (2018). Seismic Performance Assessment of Buildings, P-58-1, FEMA, Washington, D.C.
International Code Council. (2021). 2021 International Building Code, ICC, Country Club Hills, Illinois.
Other resources
Earthquake Engineering Research Institute. (2019). Functional Recovery: A Conceptual Framework with Policy Options, EERI, Oakland, California.
Federal Emergency Management Agency. (2021). Recommended Options for Improving the Built Environment for Post-Earthquake Reoccupancy and Functional Recovery Time, FEMA P-2090/NIST SP-1254, January 2021.
National Institute of Building Sciences. (2020). Resilience-Based Design and the NEHRP Provisions. NIBS, Building Seismic Safety Council, February 2020.
Want to know more about this topic? Join Justin for a free webinar on Wed. Nov. 29 at 3 p.m. ET.
This article first appeared in the November/December 2023 print issue of Civil Engineering as “Designing Differently: Integrating Resilience into Structural Design.”