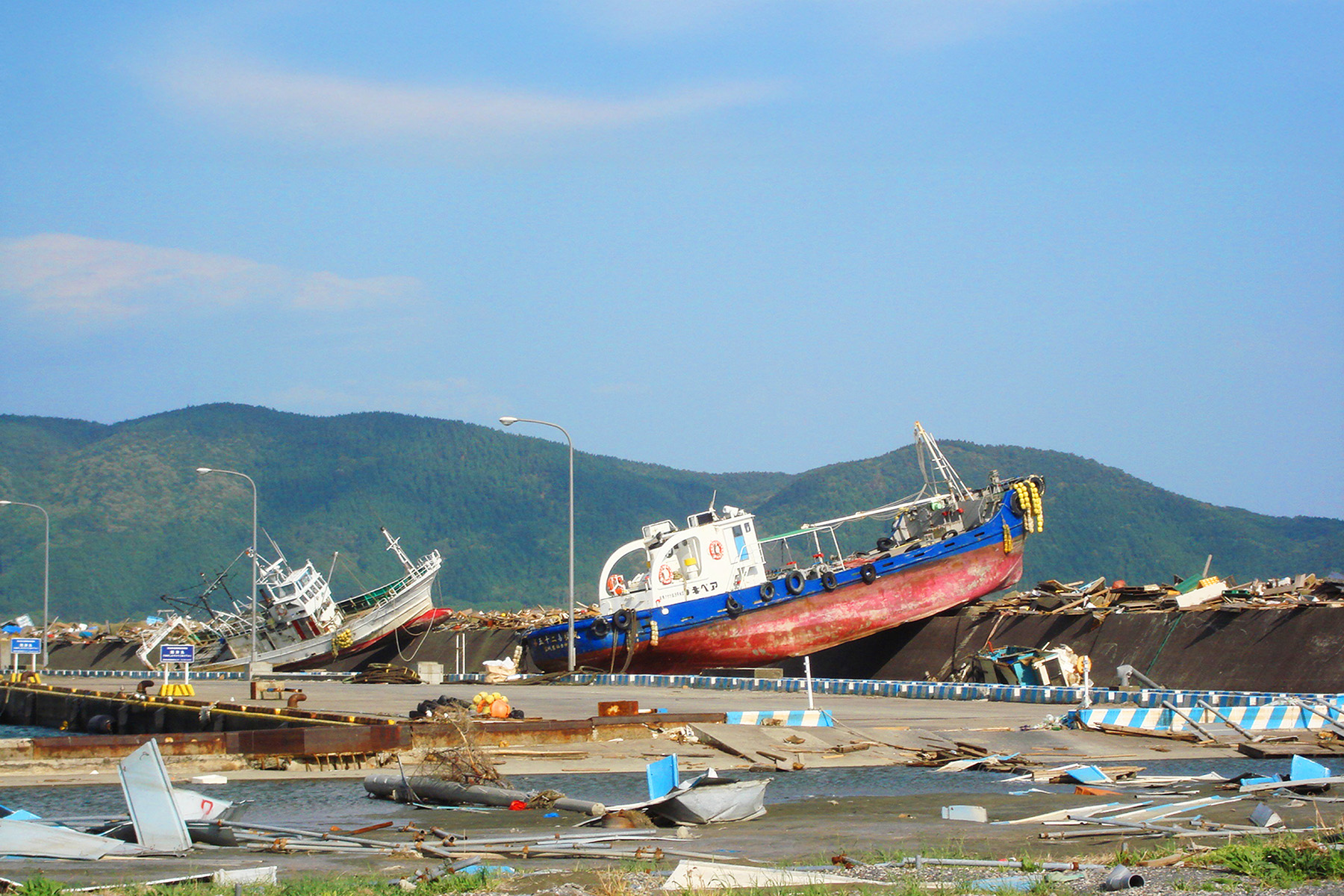
By Julie A. Galbraith, P.E., M.ASCE, and Marc I. Percher, P.E., M.ASCE
The seismic design of piers and wharves has evolved from traditional elastic analysis to more detailed nonlinear analysis. And with the advent of performance-based methodologies, civil engineers can design more economical, efficient, and resilient marine structures.
In May 2023, we arrived in İskenderun, Türkiye, as part of an earthquake reconnaissance trip for ASCE’s Coasts, Oceans, Ports, and Rivers Institute. Our work was to observe and report on the damage after the devastating February 2023 Kahramanmaraş earthquakes, which caused widespread damage and tens of thousands of fatalities.
Our first stop was once a ferry terminal, but it was most recently used as a public fishing pier. As we approached the waterfront on foot, we were taken aback by the immediate damage to a space that had been so important to the community. The transition from sea to shore was muddled and muddy due to ground settlement. Decorative teal blue railings led unnaturally to a large pool of dirty water. A wide stretch of gentle, lapping water stood where the ferry terminal entrance ramp would normally have met dry land. The site had clearly settled, a symptom of soil liquefaction seen throughout İskenderun, causing damage to the structure at the interface.
The scene was unforgettable, but marine structures can experience much worse earthquake damage. From previous reconnaissance trips and industry experience, we have seen the horrible effects of these seismic events. We have seen sites where the soil backlands had moved several feet toward the sea and caused fissures large enough to trap a pickup truck. We have visited locations at which entire wharves had collapsed into the water, piles were completely ruptured at the deck, and ships had been picked up by tsunamis and placed, ironically, on top of the marine structure.
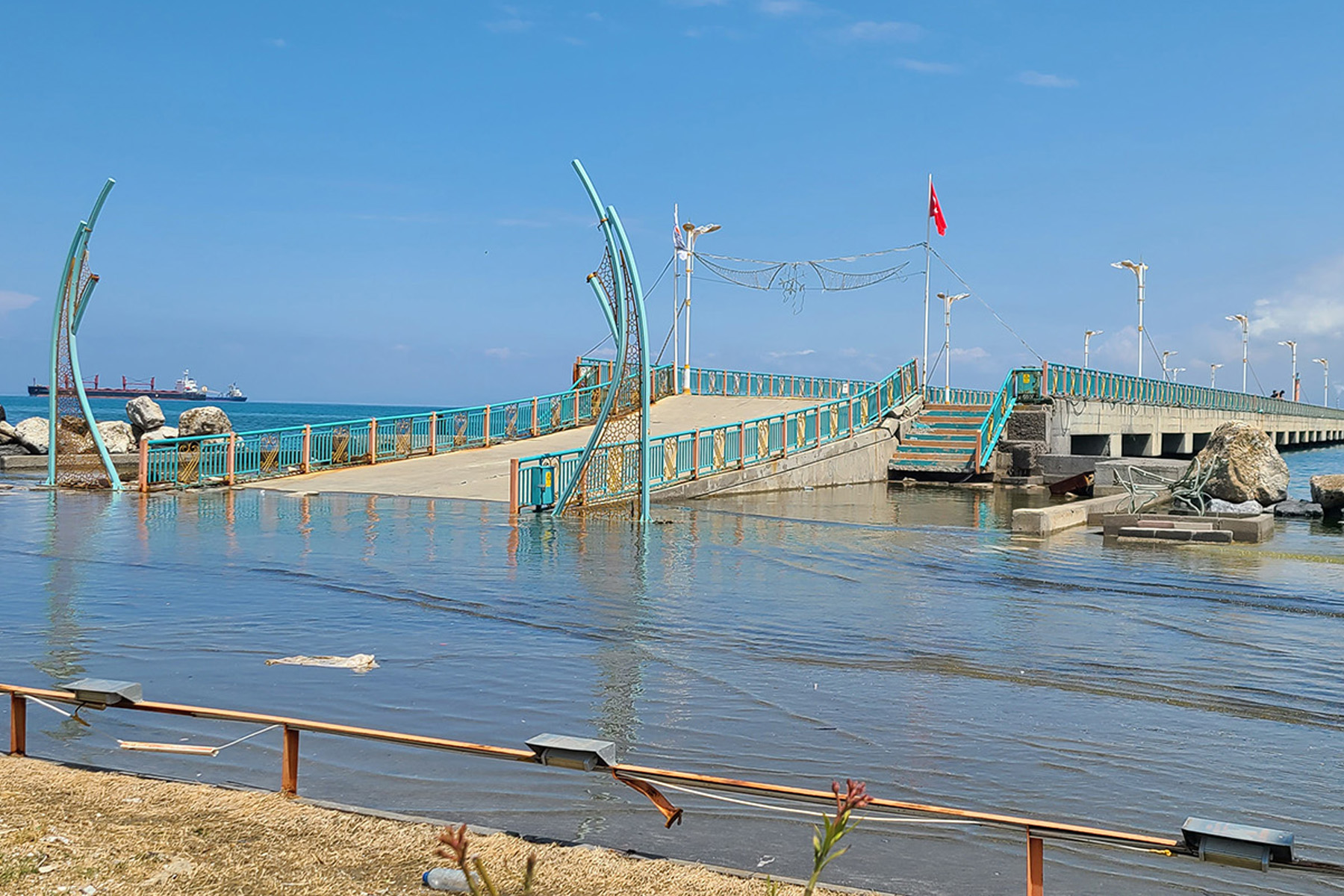
Considering these examples, it is clear that — although earthquakes’ locations and severity may vary — the primary issues of soil instability and ductile detailing are constant throughout waterfront infrastructure. The seismic design of marine structures has made incredible advances over the last few decades, in part due to the lessons learned on these reconnaissance trips, and those advancements have been incorporated into ASCE 61, Seismic Design of Piers and Wharves.
Understanding ASCE 61
Piers and wharves are unique structures with special challenges that require their own approach to seismic evaluation and design. The committees for ASCE 61 and other marine-related guidelines consider several characteristics that strongly affect seismic response and design for piers and wharves:
- Performance-based design and economic risk. Piers and wharves are often economically important to their communities (and sometimes serve as lifeline structures), which means that owners often desire a performance-based design approach that considers return to operations and potential economic loss.
- Slope stability. Piers and wharves are often constructed as bulkheads or on sloping ground; therefore, the stability of the geostructural system is critical in the seismic response. Large lateral soil movements induce high kinematic loads on piles, which has historically led to severe damage.
- Displacement control. Traditionally, piers and wharves see large deflections and lateral movements that impact supported equipment and operations.
- Soil-structure interaction. Soil conditions strongly influence the stiffness of pile-supported structures, and that stiffness is highly nonlinear in nature. Therefore, system stiffness for seismic response is also nonlinear.
- Single-degree-of-freedom response. Typically, piers and wharves have most of their seismic mass captured in the first mode of vibrational response (as the deck swaying drives inertial motions), which makes simplified SDOF methods appropriate.
- Weak column/strong beam approach. Similar to highway bridges (and the opposite of buildings), the pile-to-deck connection is conventionally the point of significant ductility and damage, whereas the deck diaphragm remains elastic. This is desirable to control damage to a repairable location and ensure post-event deck operability. Capacity protection of the deck ensures that this behavior will occur.
The first piers and wharves standard (ASCE 61-14) recognized the importance of these characteristics. The second edition (ASCE 61-25) will be published this year and debuted at COPRI’s Ports ’25 conference. This article discusses key aspects of the seismic design of piers and wharves, including upcoming changes in ASCE 61-25.
Types of marine structures
There are many types of marine structures, serving a variety of roles within the community. These include public and private uses of all scales: small fishing piers, coastal seawalls, cruise terminals, oil and break-bulk loading platforms, container terminals, and naval facilities are all part of the nation’s marine infrastructure. ASCE 61-14 focused on the new design of pile-supported structures without public access when they are not regulated by other more stringent standards (such as liquid natural gas or naval facilities). ASCE 61-25 will include sheet pile bulkheads as well.
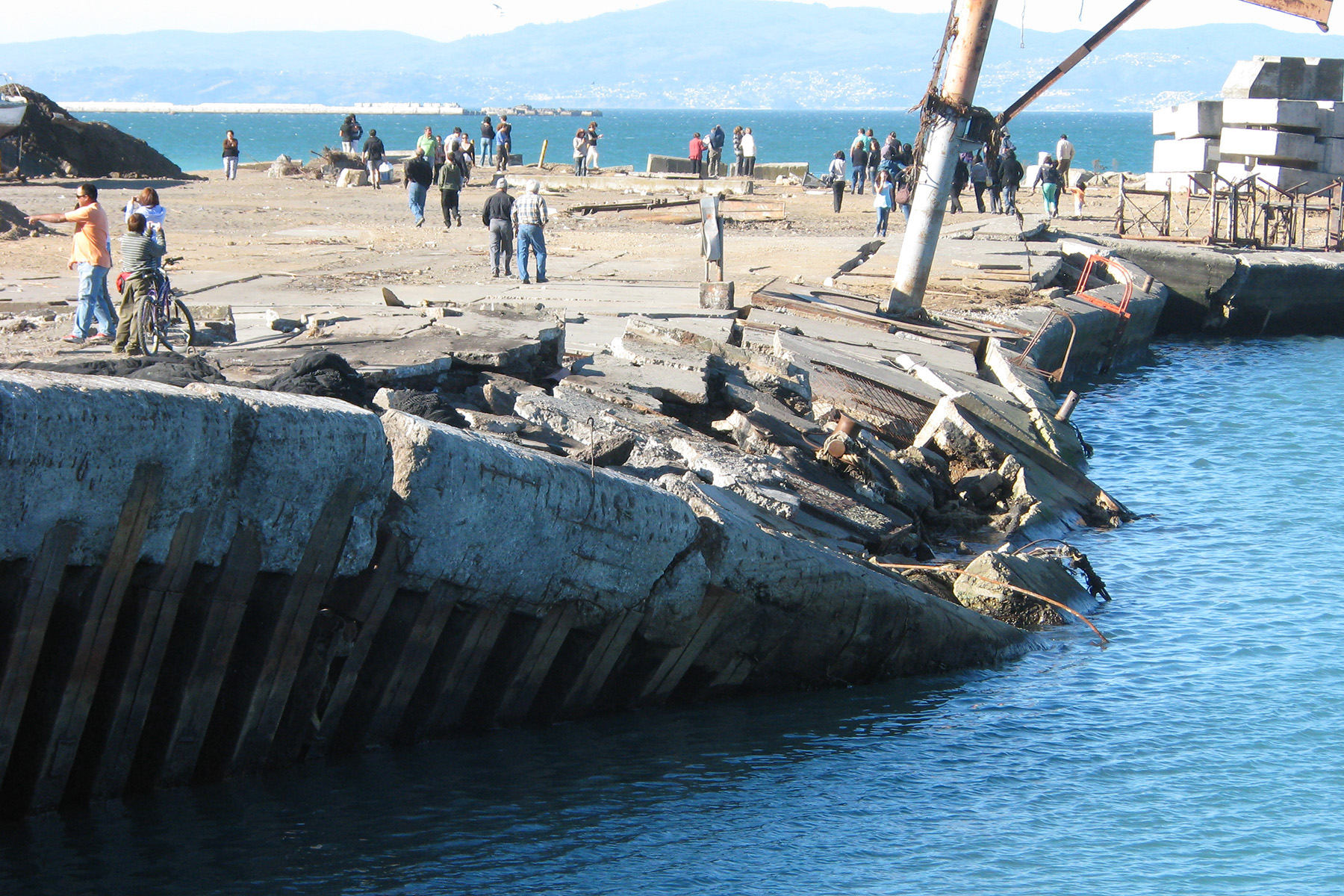
Pile-supported piers and wharves typically consist of a deck diaphragm supported directly on piles that are driven into the soils or to bedrock. Sometimes, a framing system with rows of beams or pile caps is used between the deck slab and piles. A wharf is oriented with vessels aligned parallel to the shoreline. A pier is oriented with the vessel perpendicular to the shoreline.
For design of new pile-supported marine structures, the piles control the seismic response with yielding mechanisms intentionally located at the top of the piles for a controlled inelastic response. This is the opposite of a typical building design, where beams are designed to yield first in an earthquake.
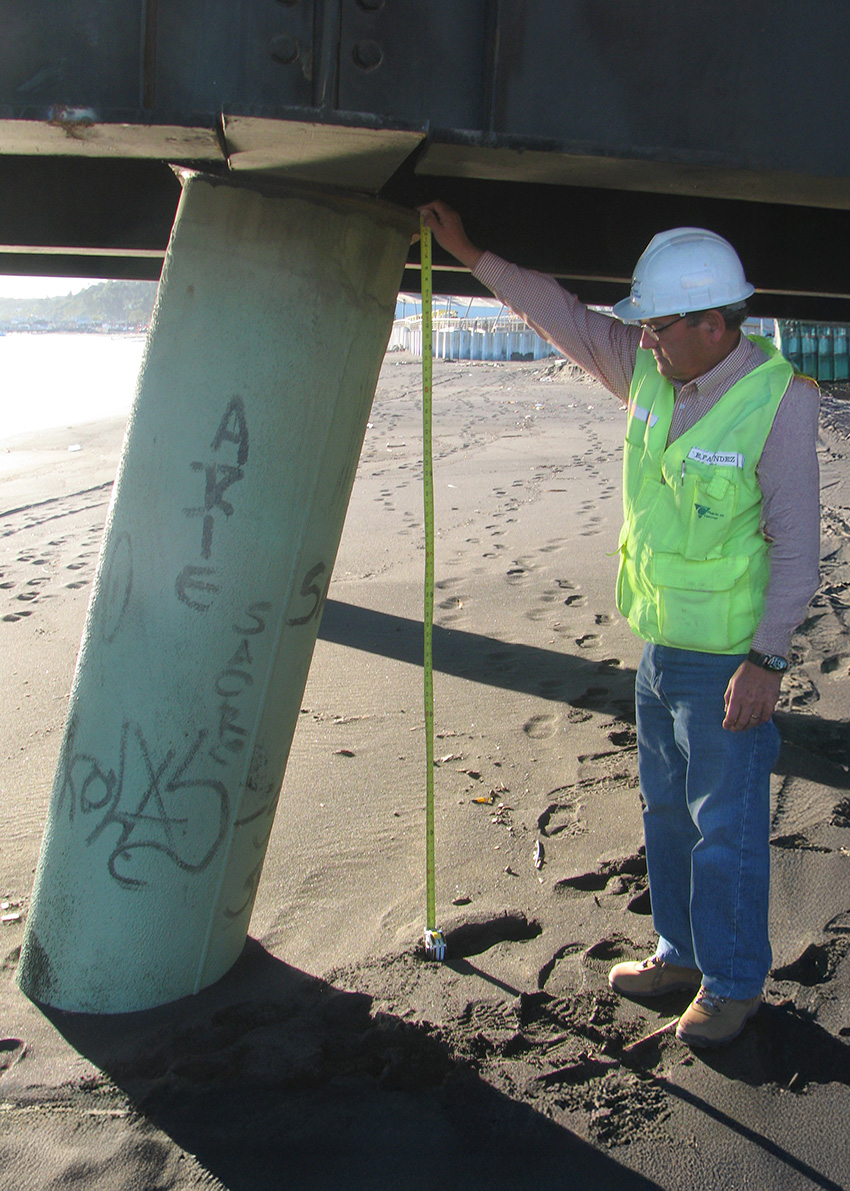
Where slopes occur, the shortest and stiffest piles will attract seismic load and may see severe damage. However, most marine structures are highly redundant and have the ability to sustain significant damage to any piles without collapse.
Bulkheads or quay walls are very common port structures and come in many forms, from earthen (berms, revetments, gravity block walls) to geostructural (cellular walls, sheet pile walls, caissons). Since bulkheads are new to ASCE 61-25, the committee has decided to focus on sheet pile bulkheads with and without tiebacks, which is a very common system in the United States in high-seismic regions.
Evolution of seismic design
Seismic analysis of pile-supported structures has changed significantly over the last 40 years, with a transition from elastic, force-based analysis to nonlinear displacement-based methodologies. From the inception of marine structure design through the 1980s, equivalent lateral force methods were the standard of practice, and design was predominantly based on provisions by the American Association of State Highway and Transportation Officials.
Seismic demands typically included a single level of uniform lateral acceleration (such as 10% to 15% of the weight of the structure). Major ports on the West Coast also developed their own criteria and followed the equivalent lateral force methodology.
In the mid-1980s, probabilistic seismic hazard analysis became the dominant approach, facilitating the use of multiple hazard levels (or earthquake severity) that are tied to a probability of exceedance (or frequency, referred to as a return period). For example, at the Port of Oakland, in California, design criteria considered earthquake return periods of 72 years (50% in 50 years) and 240 years (20% in 50 years). At that time, each hazard level had its own assumed ductility or risk factor similar in purpose to the response modification factor, R, used in ASCE 7, Minimum Design Loads and Associated Criteria for Buildings and Other Structures.
Although the standard method to evaluate seismic behavior at marine structures had evolved to consider multiple hazard levels on the demand side, the structural evaluation still used nominal design capacities for all structural elements. This general approach with force reduction factors continued through the 1990s and early 2000s, with some projects including a third hazard level.
Performance-based seismic design of waterfront structures began in the 2000s. This first occurred with the publishing of Seismic Design Guidelines for Port Structures by the World Association for Waterborne Transport Infrastructure, or PIANC, in 2001 (both authors are also on PIANC Working Group 225, which is updating this document).
Around this time, the California State Lands Commission also developed Marine Oil Terminal Engineering Maintenance Standards (MOTEMS). First published in 2002, this comprehensive document provides operational, mechanical, electrical, and structural requirements — including seismic evaluation and design — for new and existing marine oil terminals in California. These requirements were adopted by the California Building Code and took effect in 2006, with jurisdiction over more than 30 waterfront structures in California.
The MOTEMS criteria include two seismic hazard levels, with different performance targets intentionally tied to repairability and operational downtime. The Level 1 earthquake, with a shorter return period and lower design acceleration, generally causes only minor structural damage and minimal to no loss in operations.
The more severe Level 2 earthquake allows for controlled inelastic behavior and temporary loss of operations, provided there is no collapse or oil spill. This multitiered approach was becoming widespread, at least along the West Coast, as individual ports began to adopt similar or identical performance-based standards. However, the jurisdictional requirements were inconsistent. Many piers and wharves would fall into design criteria gaps because they were neither a California oil terminal nor within a major port jurisdiction. A national standard for the seismic design of marine structures was needed.
The first standard: ASCE 61-14
Luckily, industry leaders saw this need coming, and in 2005 an ASCE standard committee was formed. More than 40 engineering professionals — including owners, regulators, consultants, and academics — collaborated to develop a minimum design standard, culminating in ASCE 61-14. The first edition used a performance-based design approach, like MOTEMS.
However, ASCE 61-14 defines up to three hazard levels and performance states based on the structure’s design classification, or importance. Those with a high design classification, such as facilities essential to the regional economy or post-event recovery, are designed for all three levels. Low design classification structures, on the other hand, only need to satisfy life safety protection at the design level earthquake as part of ASCE 61-14.
The development of these performance-based design standards was an important step in advancing the marine industry. These standards recognize that marine structures behave differently from buildings, with specific failure mechanisms, inherent redundancy, and unique seismic demands (like ground failures) that cannot be linearly scaled based on earthquake magnitude. Further, the performance-based approach acknowledges that many waterfront structures are critical assets that need to not only provide life safety in a large earthquake but also withstand a moderate earthquake with minimal damage and downtime.
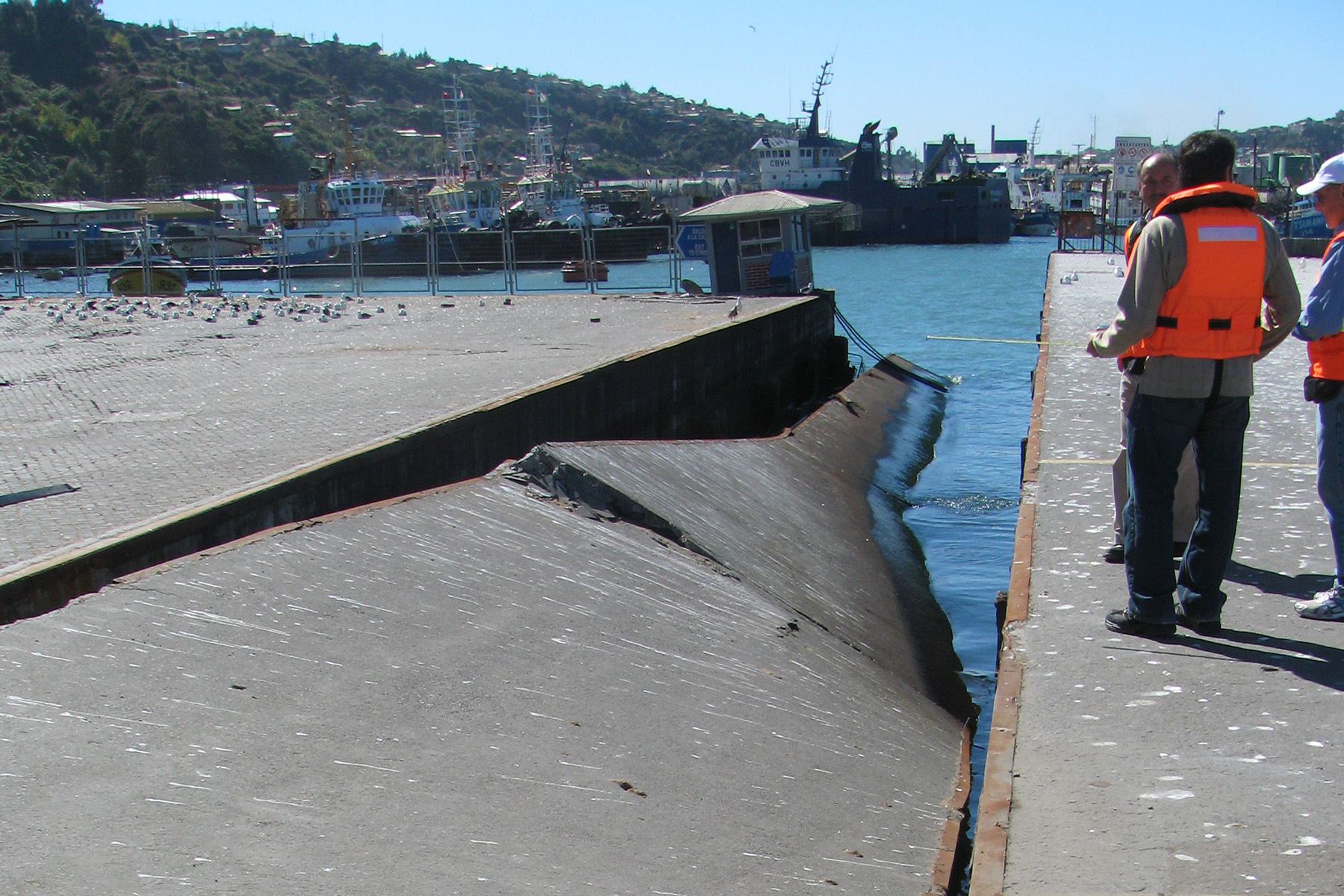
It should be noted that ASCE 61-14 includes force-based design methods, but they are permitted only for low design classifications, for low design accelerations (SDS<0.33), or for any classification and acceleration if the structure remains elastic with an R of 1.0. It is uncommon to use force-based methods in high-seismic regions. Owners typically desire an economic design at various performance design levels.
Seismic analysis for multiple performance levels is achieved through nonlinear static (pushover) methods. ASCE 61 provides different seismic design events for operation, contingency, and life safety performance levels and associates specific performance objectives (levels of damage) with each design event.
This approach uses nonlinear soil springs and nonlinear hinges at critical locations in the structure, incorporating code-defined material strain limits. For example, at the pile-to-deck connection, the engineer performs a section analysis to develop a moment-curvature relationship. Then, using nonlinear hinges, the engineer can represent the post-yield behavior of the section in the structural analysis model.
Therefore, as the pushover analysis is performed, the engineer can evaluate the progression of damage and identify the code performance limits relative to global displacement. This process defines a global displacement capacity at each performance level. Displacement demand is calculated based on system stiffness, mass, and acceleration at an equivalent or effective stiffness that accounts for system damping and degradation. The system is considered acceptable when displacement capacity exceeds displacement demand for all performance objectives.
The second standard: ASCE 61-25
For the second edition, the ASCE 61 committee worked collaboratively and diligently to improve and broaden the scope, clarify performance requirements, and add (or modify) technical requirements based on current practice, new knowledge, or other industry experience.
There have been a number of improvements. Starting with the overall scope of the document, as mentioned earlier, the committee added a chapter on steel sheet pile bulkheads. Further, the committee has revised the introductory language to remove the exclusion of structures with public access, and it no longer explicitly refers to “new” design.
Since the publication of ASCE 61-14, numerous authorities having jurisdiction have approved the use of all or part of the document for applications beyond its original intent, such as evaluating existing structures. Although intended for new design, the standard is useful for other applications, so the change in language acknowledges this and provides flexibility for AHJs to approve other applications on a case-by-case basis.
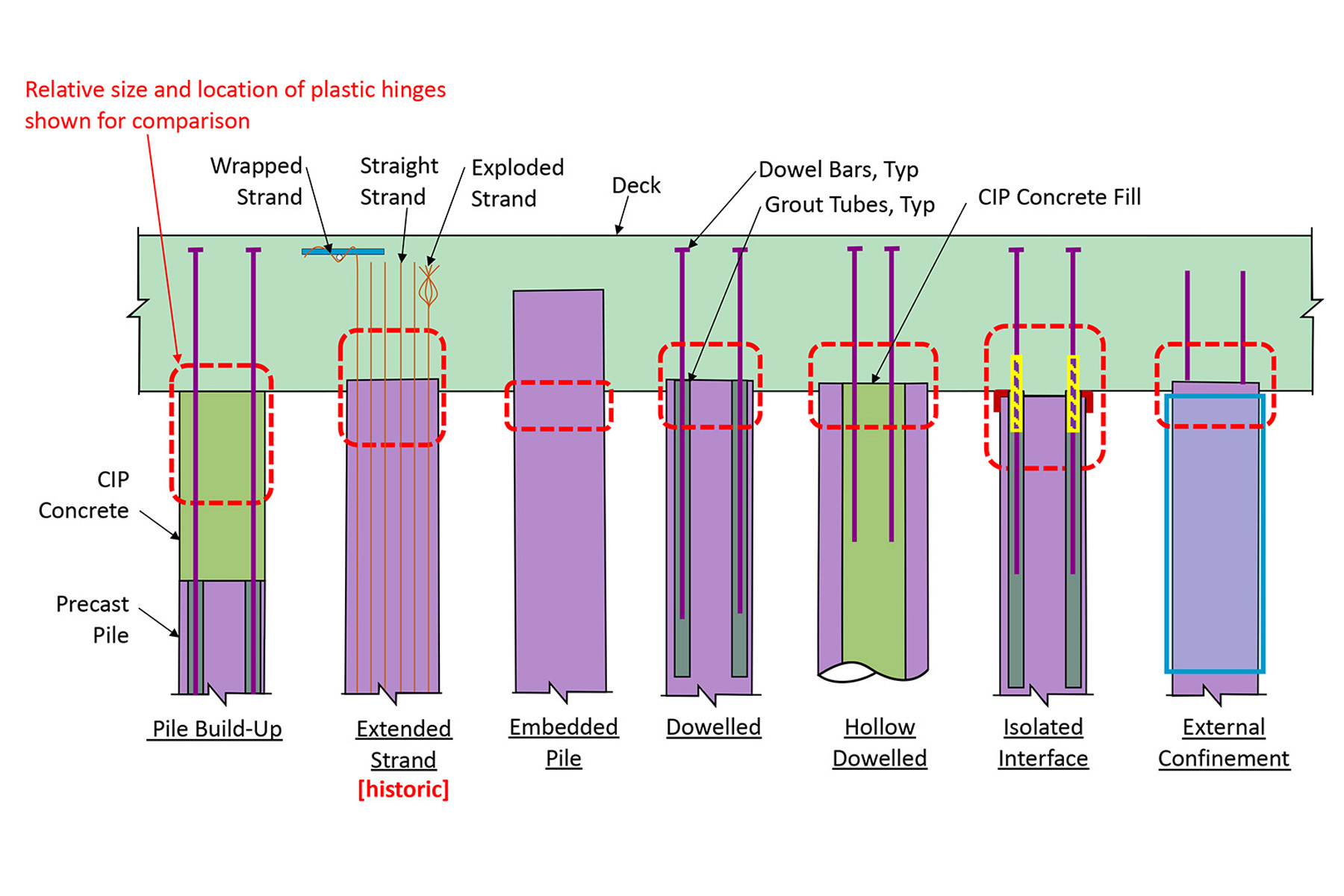
Also, the minimum seismic hazards and performance objectives have been refined in ASCE 61-25. Most notably, the largest demand event is now the life safety earthquake, taken as a 975-year return period, and the design event earthquake as defined by ASCE 7 is now used for the collapse prevention performance check (only when required by the AHJ). This shift to LSE at the 975-year hazard is more consistent with AASHTO, reflecting the fact that marine structures are more similar in configuration and response to bridges than to buildings. Also in Chapter 2, the “Design Classifications” have been renamed to the more appropriate “Importance Categories.”
The design approach in ASCE 61-25 is largely the same as in the first edition; however, the committee made important additions. Updates within Chapter 3 include new language to address torsional effects, equations to determine live load mass contribution, minor refinements to strain limits, and a footnote to consider local buckling of steel piles.
The hollow or sand/soil-filled steel pile strain limits are recognized as possibly susceptible to local buckling and requiring careful consideration by the designer. The committee was able to facilitate physical testing research on this topic and anticipates incorporating testing findings in future editions. For those interested in the steel buckling and strain limits topic, there is a detailed discussion within the updated standard’s commentary.
There have also been improvements throughout that clarify requirements or align with best practices. Examples of these changes include updated language on orthogonal loading, the use of upper- and lower-bound soil springs, and the inclusion of the coefficient method for determining demand displacements. The committee also added details on the development of ground motions, including a focus on multiperiod spectra, and requirements for peer review in certain instances. Chapter 7 includes several refinements to connections and other detailing provisions and figures.
Requirements for bulkheads are based on developments within the future bulkhead design guidelines. For now, the standard addresses only sheet pile systems, recognizing that these systems are very prevalent in U.S. ports.
Bulkheads must provide adequate resistance to static, operational, seismic, and post-seismic loading situations to satisfy project-specific performance requirements. While bulkhead response is nonlinear in nature due to soil performance, the structural components of the bulkhead (sheet pile, tieback, etc.) are required to remain elastic. Since the bulkhead sees constant lateral earth pressure, yielding within the structural components could lead to progressive collapse of the bulkhead, which has been seen in seismic events.
Therefore, bulkhead analysis focuses on the displacement of the geostructural system, stability of the system, and forces on structural components within the system. The seismic design of bulkheads also reflects project-specific criteria (such as post-earthquake operation of the facility) that are often controlled by the combination of structural deformation and global soil displacement.
Also for bulkheads, ASCE 61-25 addresses simple screening criteria, static and pseudostatic limit equilibrium methods, soil spring methods, ground motion-based sliding block procedures for seismic displacement, and geotechnical and structural methods of nonlinear time history analysis. Displacement-compatible or strain-compatible methods of linear analysis are allowed for projects involving lower seismic demand and competent soil deposits, whereas projects with high seismic loading and soil vulnerable to cyclic degradation and deformation should incorporate nonlinear methods of analysis for geotechnical and structural bulkhead design.
Adapting to change
The seismic design of piers and wharves has advanced tremendously from traditional elastic analysis to more detailed nonlinear analysis, which can account for both the inertial ground shaking and soil instability hazards that are common for marine infrastructure. Further, the use of performance-based design methodologies allows owners to develop economical designs that satisfy the desired performance while mitigating risks at various hazard levels.
Reflecting on our reconnaissance in Türkiye, it is important to remember how our ongoing work on code committees plays an important role in the seismic resilience of marine structures. This real-world experience focuses our understanding and shapes the codes we use to design waterfront infrastructure, recognizing its complex and unique role in our society. It is our hope that the lessons learned will help all civil engineers create more resilient marine facilities in the future.
Julie A. Galbraith, P.E., M.ASCE, is an associate principal at Simpson Gumpertz & Heger Inc. in Oakland, California.
Marc I. Percher, P.E., M.ASCE, is a senior marine engineer with GHD Inc. in Oakland, California.
ASCE 61-25 at Ports ’25
Given the complexity and specialized knowledge necessary for the seismic design of piers and wharves, ASCE has developed a half-day, in-person class for the Ports ’25 conference (June 1-4 in Providence, Rhode Island), as well as a 24-hour online training course. The half-day class is considered a basic primer for owners, managers, and entry-level engineers who want to understand the key parameters involved in seismic design of piers and wharves. The online course goes into significantly more depth and is intended for engineers who will be performing the analysis and detailing. The online course is available on demand from the ASCE online training center, along with other courses addressing port engineering.